Lead Authors: Dina Rabadi1, Sarah Matatov2
Co-Authors: Carolina Guerrero1, Nina Klee1,Whitney Nicanor Mabwi3, Bamlak Messay1, Yu Quan Ng4, Melanie Prakash1, Andrew Sasser1, Jillian Troth1
Dartmouth College1, University of Illinois at Urbana-Champaign2, Moi University3, Williams College4
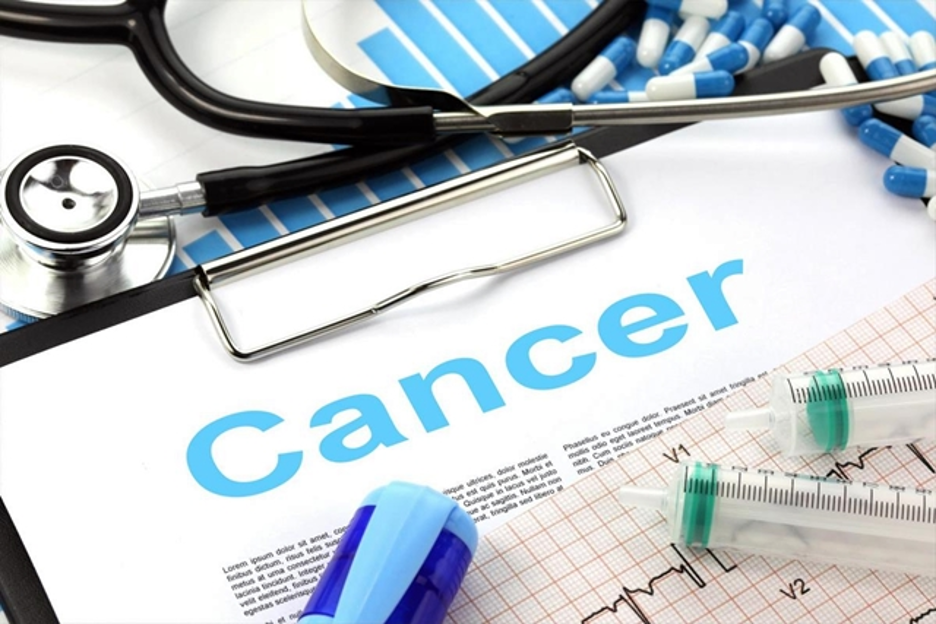
Cover Figure: A clipboard surrounded by various drugs and delivery methods to treat cancer, showing the vast options and challenges surrounding the disease (Nick Youngson, Picpedia.org).
Introduction
This article is the first of several in a series. Our objective is to provide a comprehensive overview of drug development in oncology so as to educate and inform readers on the complexity of treating cancer (which owes to the immense complexity of cancer itself), which will eventually afflict more than one-third of people currently living in the United States (“Cancer Statistics,” n.d.). Given the multi-faceted nature of cancer diagnosis and treatment, it is important to examine not only its biological mechanisms but also the history of the field of cancer research, the role of healthcare systems, the impact of pharmaceutical companies, and the emerging role of technology in the field.
The three major areas in oncology are medical, surgical and radiation oncology. While medical oncology, which includes therapies like chemotherapy or immunotherapy to treat cancer, will be the main subject of this series, we want to acknowledge alternative areas and treatments in oncology as well. Surgical oncology involves the removal of a tumor and surrounding tissue through surgery, and radiation oncology is the treatment of cancer using radiation therapy. Patients may need treatment involving a combination of surgery, chemotherapy, and radiation therapy (“Types of Oncologists,” 2018).
Surgery is most useful for solid tumors contained in one particular area, because it is a local treatment. Therefore, it is rarely implemented to treat blood cancers like leukemia or cancers that have spread throughout the body. Although surgery may be the only necessary treatment, it is most often combined with other cancer treatments to ensure that every cancerous cell is gone and to reduce the chance of the cancer . Surgery can be open or minimally invasive. While open surgery involves making one large cut to remove the tumor along with some healthy tissue, minimally invasive surgery only requires making a few small cuts to insert a thin tube with a camera (a laparoscope) for displaying the inside of the body on a monitor. Depending on the stage and type of cancer, surgery can aim to remove an entire tumor, debulk a tumor by only removing it partially, or relieve cancer symptoms such as the pain and pressure sometimes caused by a tumor (“Surgery to Treat Cancer,” 2015). Further reasons to have surgery may be to diagnose cancer, to determine if cancer has spread, or to restore the appearance or function of body parts (“What is Cancer Surgery?,” 2019).
Radiation therapy damages cancer cells by using high-energy waves or particles like x-rays, gamma rays, electron beams, or protons. This radiation causes small breaks in the DNA inside cells, causing cancer cells to die or to stop growing and dividing. Radiation therapy tends to be a local treatment by which surrounding healthy cells are harmed as minimally as possible. As a result, radiation therapy is less effective for treating cancer that has spread within the body; however, it can be used to treat various types of cancer either as the sole form of treatment or in combination with others.
Radiation therapy can be administered in three ways: (1) through external radiation, by which high-energy rays are directed into the tumor from outside the body, (2) internal radiation (brachytherapy), by which a radioactive source is placed near the tumor inside the body, and (3) systemic radiation, by which radioactive drugs are taken by mouth or injected through a vein. The aims of radiation therapy are usually to cure or shrink early-stage tumors, to prevent cancer from recurring in another part of the body, to alleviate symptoms caused by advanced cancer (palliative radiation), or to treat recurred cancer (“How Radiation Therapy Is Used to Treat Cancer,” 2019).
Chemotherapy
Overview
Chemotherapy is a type of therapy that involves attacking cancer cells on the cellular level, by killing the cancer cells. There are many different forms of chemotherapy, such as electrophilic reagents, or an attacking chemical group, reacting with cellular nucleophiles, receiving chemical groups, chemicals adding alkyl groups to DNA bases, making causing DNA damage and killing the cell, the use of antimetabolites to interfere with synthesis of DNA precursors, as well as combination therapy, using different combinations of drugs. The theory is that by attacking several different targets, chemotherapy treatments can take out a wide variety of cancerous cells. One problem with this approach is that tumor cells and normal cells share many structural characteristics. As a result, chemotherapy often damages healthy cells in addition to cancerous ones (Shewach & Kuchta, 2009). Many studies, such as one done by Mannion et al., report increased quality of life among patients who have undergone chemotherapy (Mannion et al., 2014). However, chemotherapy, like many other cancer therapies, can have detrimental side effects that are the result of off-target effects of the drugs (Bakker et al., 2015). As with any treatment, weighing the costs and benefits is key in determining which approach might be best for a patient.
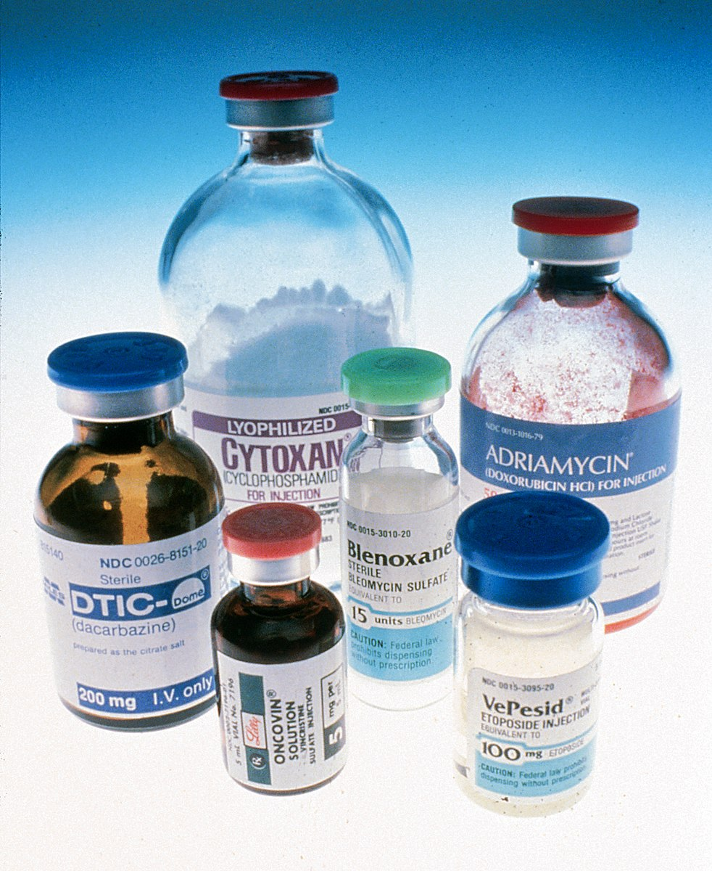
Figure 1: Bottles of chemotherapies that were used in the United States circa 1993 (Source: Wikimedia Commons, National Cancer Institute).
Chemotherapy is used in conjunction with surgery and radiation therapy to stop cancer cells from dividing uncontrollably. Other conditions that chemotherapy can also treat include bone marrow diseases, in which chemotherapy can prepare the patient for a bone marrow transplant, and hyperactive immune system disorders such as lupus and rheumatoid arthritis.
Successful chemotherapeutic treatment is about balance: distributing a regimen strong enough to defeat the cancer without also defeating the patient. New philosophies for chemotherapy distribution seek to adhere to this idea. For example, metronomic chemotherapy, as seen in Figure 1, requires frequent, regular administration of drug doses. The goal of this strategy is to use a sort of low-intensity warfare, keeping low but active concentrations of drugs in the patient’s system for long periods of time. One reason this is done is in hopes of preserving Quality of life (QoL), which is defined by the World Health Organization as the comparison of a person’s current life in comparison to the life they had thought they would or wish that they do live (“What Is QOL?,” n.d.).
Another factor to consider is that different concentrations and schedules of chemotherapy can affect anti-tumor action. Especially with combination therapy, the effects of chemotherapy on cancer can vary (“Pharmacokinetics of Metronomic Chemotherapy,” n.d.). Generally, standard chemotherapy looks to administer a “maximum tolerated dose”, but this doesn’t address cancer’s nature as a “chronic disease” because versions of cells that can outlast the initial chemotherapy could still persist (Scharovsky et al., 2009). Metronomic chemotherapy requires 2-3 week breaks between successive cycles, and it is administered at very high doses that can often have maladaptive effects and may not be tolerated by some patients. In some models, metronomic chemotherapy can be effective in cancer cells that have resistance to those very chemotherapeutics. (“The Anti-Angiogenic Basis of Metronomic Chemotherapy,” n.d.).
Chemotherapy treatments can also vary in pediatric conditions. Because so many signaling patterns coexist in the child as they grow, receiving chemotherapy can be extremely dangerous because it may affect other biological pathways. Another problem is that children who are on a pediatric oncology ward might not be able to communicate their tolerance of a specific regimen, and their QoL can sink dramatically as a consequence (“Palliative Care in Children with Cancer,” n.d.). Additionally, children are in a state of constant and rapid growth. Healthcare Providers and cancer scientists need to account for varied biochemical compositions, such as variations in signaling pathways, when designing chemotherapy schedules (“Palliative Care in Children with Cancer,” n.d.).
Advantage and Disadvantages
One of the greatest advantages of chemotherapy is its applicability in the treatment of many different cancer types; the different forms of chemotherapy include curative, adjuvant, neoadjuvant, and palliative. Curative chemotherapy is implemented exclusively or primarily to eliminate all cancer cells. Adjuvant chemotherapy is used to fight hidden cancer cells that could be left in the body after surgery or to prevent future recurrences. Neoadjuvant therapy is effective for treating sizable tumors that cannot be directly operated on; the therapy functions by shrinking the tumor enough to enable treatment via radiation and surgery. Lastly, palliative chemotherapy is used to relieve symptoms or improve the quality of life of the patient for terminal forms of cancer.
Since chemotherapy attacks all rapidly dividing cells, the cytostatics (drugs that target cell growth) can also attack healthy cells, such as red blood cells. This drop in red blood cell count can weaken the immune system, causing a variety of short-term side effects. Hair loss, anemia, nausea, vomiting, diarrhea, and infections in the mouth are all common side effects that can occur as a result of chemotherapy treatment. However, these side effects can be prevented or relieved through other treatments. For example, blood transfusions can help ease the effects of anemia, anti-nausea drugs can relieve nausea and vomiting, and infection can be prevented with antibiotics or antifungal drugs. The long-term side effects of chemotherapy often emerge months or years after treatment, and these effects can depend on the type of chemotherapy treatment that the patient received. Damage to lung tissue, heart problems, infertility, nerve damage, and recurrence are all possible long-term effects that can threaten the patient’s health.
Alkylating Agents
One of the first types of chemotherapy drugs to be developed historically were the so-called alkylating agents. Derived from mustard gas—a toxic gas first used in World War I as an agent of chemical warfare—alkylating agents were introduced as chemotherapeutic agents in 1946 (Brookes, 1990). Early studies of these compounds found that they were incredibly effective against a wide variety of cancers, particularly lymphomas; however, they were often non-specific and could cause tremendous side effects if given in high doses (Brookes, 1990). Ultimately, further study produced much more useful compounds, such as cyclophosphamide and busulfan, which continue to be used as chemotherapeutic agents today (Colvin, 2003).
Alkylating agents, which are typically composed of electron-rich substituents, act by forming covalent bonds with DNA. Most alkylating agents work by transferring an alkyl group—a chemical substituent made entirely of carbons and hydrogens—to a DNA molecule (Prendergast & Jaffee, 2007). This inhibits the transcription of a cancer cell’s DNA to its corresponding RNA, thereby inhibiting its translation to the proteins that it requires to grow and replicate. The effectiveness of alkylating agents is mediated by the ability of the cell to recognize and repair the DNA damage they cause (Prendergast and Jaffee, 2007).
In modern treatment, there are two main classes of alkylating agents. Classical alkylating agents, like cyclophosphamide and melphalan, attach a methyl group —a central carbon with three connected hydrogen atoms —directly to DNA (Colvin, 2003). Typically, these agents are not bioactive until introduced in the body; from here, they can decompose into reactive groups like chloroethylaziridine that alkylate DNA. Classical agents have been used to treat multiple myeloma, ovarian cancer, and breast cancer (Colvin, 2003). The other common type of alkylating agents are the “alkylating-like” agents. These complexes, which do not actually affix an alkyl group to DNA, still operate in the same way, with platinum metal complexes coordinating to DNA and hindering its ability to be transcribed (Colvin, 2003). Examples of these compounds include cisplatin (used against testicular cancer) and its less cytotoxic variant, carboplatin (Colvin, 2003).
Mitotic agents
For a long time, anti-mitotic agents have been used to fight cancer, as they target the crux of the disease: abnormal mitosis or cell division. There are different checkpoints in the process of mitosis to prevent abnormally formed cells from multiplying. Some of these checkpoints fix the issue present in the premature cell, by slowing down the cell cycle and stopping the cell from proliferating into a tumor, while other checkpoints initiate cell death to prevent abnormal cells from proliferating.
Microtubule-targeting agents (MTAs) are common antimitotic agents and function by targeting kinase pathways. MTAs trigger the spindle assembly checkpoint (SAC) to hold the cells in a static, non-dividing state, so as to ensure that chromosomes segregate correctly and that mitosis creates identical daughter cells (Dominguez-Brauer et al., 2015). MTAs also work to inactivate the dynamics of microtubules so that cell death eventually occurs. MTAs are relatively effective in preventing the metastasis (spreading) of some cancers; however, they can also cause harmful side effects, and can result in toxicity and cancer resistance to the drug.
Anti-kinase agents are a class of drugs that target entry and mitotic kinases. Entry kinases are responsible for leading cells into the mitotic phase of the cell cycle. The Cdk1 kinase, for example, is part of a complex that initiates mitosis, so when it is inhibited by a drug, it causes the cell to die. The theory that Cdk1 kinase would be a good cancer treatment has not held up in clinical trials, however, as the drug has proven to have significant harmful side effects. There are other kinases like Chk1 and Chk2, which are checkpoint kinases, that have been used as drug targets, but these kinases demonstrate negative side effects that outweigh their limited efficacy as well (Dominguez-Brauer et al., 2015).
Anti kinase agents have the ability to act on mitotic agents, but they are of the Aurora kinase and polo-like kinase (Plk) classes. Aurora kinases tend to be overexpressed in many cancers, and consequently scientists have target them using a cocktail of drugs. Plks are also overexpressed in many cancers, and thus specific Plks have been identified as targets for anti-kinase agents. In particular, inhibition of Plk1 and Aurora A kinases has been shown to induce cell death. The various types of Plks and Aurora kinases affect different parts of mitosis. Anti-motor protein agents target kinesins, which are proteins that help the cell transport molecules down microtubules, structures that make up the skeleton and “transportation highway” of the cell. Research suggests that the drug Monastrol was able to effectively inhibit the mitotic kinesin, Eg5, firmly preventing cancerous cells from progressing in the process of mitosis. This finding has encouraged scientists to explore kinesins further as drug targets. While drugs like Monastrol have proven to show no toxicity to cells and are associated with minimal drug resistance, the cancer finds another path through which to proliferate, they have not yet been tested extensively in clinical drug trials (Chan et al., 2012).
Antitumor Antibiotics
Antitumor antibiotics, also known as anticancer antibiotics and antineoplastic antibiotics, are a class of anticancer drugs that block cell growth by interfering with DNA. Three DNA-damaging metabolite families are considered antitumor antibiotics: Enediynes, Mitomycin, and Bleomycin (Galm et al, 2005). In 1985, enediynes were the first class of antitumor antibiotics to be discovered. These antibiotics are derived from bacterial sources and work by self-activating, they activate when they enter a certain chemical environment, three functional domains allow them to cause DNA damage. The first domain is a “warhead” which “explodes” when activated and damages DNA. The second is a delivery system that can deliver this “warhead” to its target. The third is an activation device that is specifically triggered and then itself triggers a cascade that leads to activation of the warhead. Using these three mechanisms, the antibiotic molecules can enter and damage cancer cell DNA (Nicolaou & Dai, 1991).
Mitomycins are a class of antitumor antibiotics that have been researched for their DNA damaging abilities for over 30 years. In particular, one subtype of the mitomycin family, mitomycin C (MMC), has been widely investigated in the context of cancer therapy. MMC works by inhibiting the synthesis of DNA. Because cancer cells replicate DNA and divide at a much faster rate than healthy cells, they are therefore more vulnerable to this treatment. Oxidized MCC is inactive and can be activated by enzymatic reduction. Once active, MCC binds DNA with either mono- or bi- functional alkylation, or the addition of a carbon group. When DNA is bifunctionally alkylated, it is crosslinked to MCC and cannot be used for replication, leading to eventual death of the affected cell (Verweij & Pinedo, 1990).
Bleomycin antitumor antibiotics, another type of antibiotic, produce double-strand breaks in DNA at specific sites. These breaks are likely a result of two independent single-strand breaks at nearby sites on opposite strands of the DNA. Bleomycin antibiotics generally cleave DNA at GC and GT sequences, and occasionally at AT sites. This cleavage activity is also enhanced by reagents like 2-mercaptoethanol and ferrous ions. Ferrous ions are actually such a potent supplement that in their presence bleomycin cleaves DNA at TT, AT, and TA, as well as at GC and GT sequences (D’Andrea & Haseltine, 1978).
Finally, anthracyclines are a modern class of antitumor antibiotics that are widely used as a chemotherapy treatment. Anthracyclines are extracted from Streptomyces bacteria, and they interfere with enzymes involved in DNA replication during all phases of the cell cycle. They are widely prescribed for a variety of cancers and include common chemotherapy drugs such as Daunorubicin, Doxorubicin (Adriamycin®), Epirubicin, and Idarubicin (Longdom Publishing, 2021).
Overall, antitumor antibiotics are effective in their treatment of cancer. The drugs are delivered to the body intravenously and then enter cells, damaging DNA and therefore killing cancer cells. There are some concerns with this method as the DNA damaging abilities of the antibiotics can also damage healthy cells, specifically increasing the risks of permanent heart and bone marrow damage. To combat these risks, many of the treatments are only allowed at maximum time intervals. For example, MCC is only given every six weeks, and dosages are carefully controlled (Longdom Publishing, 2021).
Topoisomerase inhibitors
Topoisomerases inhibitors are a class of chemotherapy that targets the function of Topoisomerase enzymes. Topoisomerase enzymes are responsible for controlling, maintaining and modifying the topology of DNA during replication and transcription. Topoisomerases are separated into two classes of enzymes: I and II. While many of their functions are the same there are a few key differences: Topoisomerase I (Topo I) is not a cell cycle specific enzyme whereas Topoisomerase II (Topo II) is highest in log phase, a phase of the cell cycle where cell growth is defined by a logarithmic curve, and replicating tumors. Topo I only induces single stranded breaks whereas Topo II induces both single and double stranded breaks (Sinha, 1995). The fact that Topo-II is a cell cycle dependent enzyme makes Topo-II inhibitors less desirable as an anti-cancer therapy. But Topo-I inhibitors have proven successful.
Topoisomerase I Inhibitors were first discovered in extracts from the plant species Camptotheca accuminata, which had significant anti-tumor activity in leukemia. Later, camptothecin was discovered to be the active agent in this extract. In order to use camptothecin in clinical trials, a more water soluble analog was synthesized: NSC-603071 (9-Aminocamptothecin). NSC-603071 successfully bound and acted on Topo I and initiated DNA damage and cell death in human trials. One drawback of this drug is that in higher doses it can lead to bone marrow and gastrointestinal toxicity, but this has been managed with dose regulations. Topotecan is another Topo I inhibitor that has been shown to be effective against a variety of cancers including refractory colorectal cancer, cancers of the head and neck, and malignant glioma. But its wide use also leads to a significant chance of hematological toxicity in the patients who take it. Finally, Irinotecan is another Topo I inhibitor that is another water-soluble camptothecin analogue that has shown significantly better activity against a wide range of cancers such as colon, breast, and small cell lung cancer as well as leukemia (Sinha, 1995).
Plant-Based Alkaloids
Alkaloids are a broad class of nitrogen-containing organic compounds found in nature. Typically produced by plants but also present in some microorganisms and animals, alkaloids are biologically-active compounds – some of which contain antitumor properties (“Alkaloids,” 2014). Plant-based alkaloids are often used as chemotherapeutic agents that prevent tumor growth by inhibiting cell division (“Types of Chemotherapy Drugs,” n.d.).
There are many different types of plant-based alkaloid drugs that act at different stages of the cell cycle; however, many are most effective during the S and M phases, during which DNA is replicated and cell division occurs, respectively (“Types of Chemotherapy Drugs,” n.d.). Three classes of mitotically-active alkaloids commonly used in chemotherapy are the vinca alkaloids, the epipodophyllotoxins, and the taxanes, which famously include paclitaxel (sold under the brand-name Taxol) (“Plant Alkaloids,” 2014). Although all of these drugs target steps of cell division, they are derived from different plants and have different mechanisms of action. For instance, Taxol binds to microtubules during mitosis, stabilizing the structures and preventing further spindle fiber formation (“Plant Alkaloids,” 2014). Without functional spindle fibers, the cell cannot equally divide chromosomes into two daughter cells and cell division halts. On the other hand, epipodophyllotoxins, such as the drug etoposide, prevent entry into M phase, the phase of the cell cycle where mitosis or cell division occurs, altogether by inducing DNA damage and inhibiting topoisomerase II during S phase, the cell cycle when the DNA is replicated (“Etoposide,” n.d.).
Plant-based alkaloids include a vast array of compounds which are instrumental in treating cancer. As such, they are the focus of many synthetic organic chemistry labs, which aim to design synthetic routes to mass-produce these compounds rather than harvesting them from plants. Exciting research in this field, from the discovery of new alkaloids to the development of new methods to synthesize them, is critical in the fight against cancer.
Immunotherapy
Overview
Immunotherapy is a cancer treatment that makes use of the immune system. These therapies can either boost the natural defense mechanisms of the immune system, enabling it to attack cancer cells more aggressively, or it can supplement these natural defense mechanisms with exogenous components. The immune system is not always able to destroy cancer cells because cancer cells evolve from normal, healthy cells before mutating into cancer cells that divide uncontrollably. Hence, the immune system cannot always recognize cancer cells as foreign cells to attack. Sometimes, the immune system may recognize cancer cells but not respond strongly enough to destroy them. In other cases, cancer cells might release substances that prevent the immune system from recognizing and attacking them. Immunotherapy serves as a means to get around these problems. Depending on the type and stage of the cancer, among other factors, immunotherapy can be used as the sole form of treatment or it can be used in combination with other types of treatment (“How Immunotherapy Is Used to Treat Cancer,” 2019). Many types of immunotherapy exist, including T-cell therapy, monoclonal antibodies, cancer vaccines, and cytokine inhibitors (“Understanding Immunotherapy,” 2020).
One benefit of immunotherapy is that it can supplement other therapies, including chemotherapy, which function better if prescribed in combination with immunotherapy. Furthermore, immunotherapy may be an effective treatment when other treatments fail; some cancers—skin cancer, for example—do not respond well to chemotherapy or radiation. Patients may experience fewer side effects than when undergoing radiation or chemotherapy, because immunotherapies only target one’s own immune system. Additionally, cancer is less likely to recur following immunotherapy, since the immune system has gained an immunological memory for attacking cancer cells (“Immunotherapy: What Patients Need to Know,” 2019).
However, a disadvantage of immunotherapy is that the stimulated immune system can also fight healthy cells (“Immunotherapy to Treat Cancer,” 2019). Patients may feel side effects such as flu-like symptoms, weight gain, or diarrhea, as well as pain, itching or swelling in the region where the medication was applied. Furthermore, immunotherapy may have a longer onset of action than other treatments. It is currently only successful for less than half of patients, and even if immunotherapy works at first, it sometimes loses its effectiveness in the later stages of treatment (“Immunotherapy: What Patients Need to Know,” 2019).
CAR T cells
The body possesses both innate and adaptive immune response mechanisms to defend against invasion by foreign bodies and the proteins they express, known as antigens, which determine their immunogenicity (ability to induce an immune response). An immune response is usually induced once the antigen’s epitope, the part that the antibody binds, binds with receptors on an immune cell (Dunn et al., 2002). A healthy immune system will regulate and eliminate mutated cells, decreasing the rates of tumorous incidences that arise spontaneously during the cell cycle. This regulation is possible because most of the cancerous cells contain defective or mutated cell receptors that segregate them from normal cells, making them unique and therefore susceptible to attack by lymphocytes, which are a type of immune cell. However, most neoplastic (cancer-related) cells express many of the same receptors as normal cells and are able to avoid lymphocytic binding and killing (Messerschimdt et al., 2016).
When the tumors evade immune action, the role of immune cells is essentially impaired, allowing the cancer cells to proliferate unchecked and eventually metastasize (spread throughout the body). For most cancer therapies, initial remission usually occurs with introduction of chemotherapeutic agents, which induce neoplastic cell death. However, with continual use, the tumors can develop resistance and become unresponsive to both chemotherapies and immunotherapies. With the advent of genetic editing tools, immunologists have been able to engineer antigen specific T cells targeting specific receptors on tumor cells. This new generation of repurposed cell lineages have been termed Chimeric Antigen Receptor T cells (CAR-T).
The process of engineering CAR-T cells involves harvesting T- cells from a patient and genetically modifying them to express a receptor that will bind to a surface antigen expressed on the patient’s own tumor cells. After infusion, CAR-T cells are able to migrate to the tumor and replicate. The earliest CAR-T cells consisted of an extracellular antigen recognition site linked via a transmembrane domain to an intracellular signaling domain. This setup worked to activate the CAR-T cells once they encountered the tumor. While effective in lysing (bursting opne) tumor cells in vitro, the clinical utility of these first-generation CAR-T cells was limited by their inability to sufficiently activate and sustain themselves while in the body. Second generation CAR-T cells, with the addition of costimulatory domains (including CD28) to the intracellular portion, have been engineered to enhance cytokine secretion, a single for inflammation and immune response, and effector cell expansion, and prevent activation-induced apoptosis and immune suppression by tumor-related metabolites (Carpenito et al., 2009; Eshhar et al., 1993).
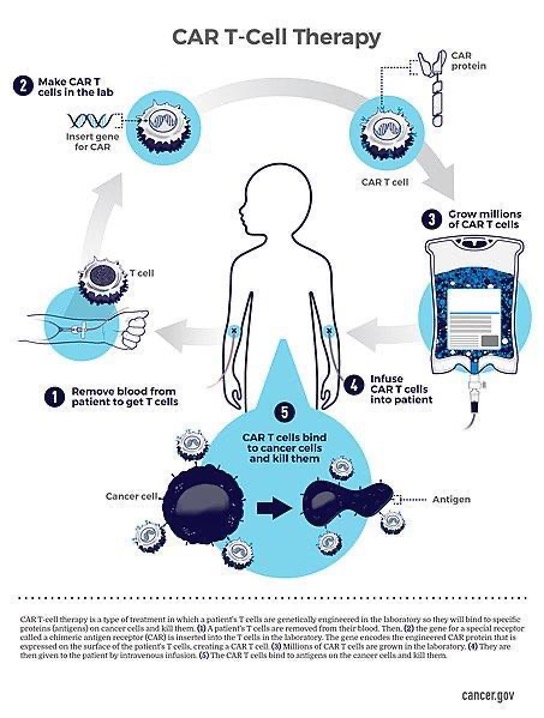
Figure 2: One process by which the CAR T-Cell process occurs. Here, blood is taken from the patient to obtain T cells, T cells are engineered in the lab to become CAR T cells, grown, then infused back into the patient to kill their cancer cells. (Source: Wikimedia Commons, National Cancer Institute).
Limitations of CAR T cells
The initial clinical remission rates after CAR-T cell therapy in all patients are as high as 90%, with remarkably durable remission rates in both acute and chronic leukemia (Brentjens et al., 2013; Maude et al., 2014; Turtle et al., 2016; Kalos et al., 2011). However, long-term survival rates are much lower. In the case of B-cell lymphomas, the cause of low long-term survival is typically the emergence of leukemia cells that do not express the protein Cluster of Differentiation 19 (CD19). The absence of this protein allows the cancer cells to evade recognition by the CD19–CAR T cells, a phenomenon known as antigen escape. Certain mutations cause CD19 protein expression to be downregulated, and thus allows the cancer to evade CAR-T cells, which are CD19 specific. Recent research endeavors are focused on generating CAR-T cells with bispecific targeting of CD19 and CD20, or CD19 and CD21. This trio of CD19, CD20 and CD21 markers are specifically expressed in both normal and neoplastic B cells, making them suitable targets. This multimodal approach would enhance both the scope of TCR-antigen cross reaction and the affinity of binding to cancer cells (Schultz & Mackall, 2019).
Currently most of the available CAR-T therapies have resulted in better prognosis in blood cancers than in solid tumors. Solid tumors present a more difficult target for CAR-T cells due to difficulties in identification of unique antigens peculiar to solid tumors. For one, selected antigens must be highly expressed on the majority of cancer cells but largely absent on normal tissues to ensure that CAR T Cell therapy is specific to the cancer – this is often not the case with solid tumors. Another problem is that a hostile micro-environment around the solid tumor can make it difficult for the CAR-T cells to bind effectively and suppress tumor activity. For example, a tumor that displays broad antigenic heterogeneity (i.e., the tumor contains many different antigens) can be difficult to target, since it is harder to recognize the right epitope when there are many to test. Furthermore, CAR-T cells are also not trafficked efficiently into the center of solid tumor masses as they preferably bind on surface antigens (Schultz & Mackall, 2019).
Several side effects resulting from CAR-T cell therapy have been documented, with the notable and severe effects being cytokine release syndrome and neurologic toxicity. In adverse cases, cross reaction occurs whereby sometimes the CAR-T cells attack off-target tissues and release granzymes and perforins, whose lytic action kills normal cells (Schultz & Mackall, 2019). Since this is a relatively new treatment option, further data regarding the therapy’s long term impacts are limited (Breslin, 2007; Hartmann et al., 2017).
Monoclonal antibodies
Antibodies are part of the body’s own adaptive immune response. They are vital in both the recognition of foreign antigens and the stimulation of an immune response to them. Once an antibody binds an antigen, immune cells such as T-cells are able to locate and attack the foreign antigens. The four intrinsic immunoglobulin subclasses, or types of immunoglobulin cells, are therefore implicated in antibody dependent cytotoxicity. Due to their unique and diverse antigen specificity, antibodies have been clinically reengineered into effective target-specific antitumor agents. They are referred to as monoclonal antibodies, and are able to recognize proteins that are overexpressed on a patient’s tumor cells relative to the rest of the body’s normal tissue. The first cultured monoclonal antibodies were synthesized in the 1970s by Kohler and Milstein after a series of modifications on the hybridoma technology (fusion of a lymphocyte with a tumor cell) which they had pioneered (Köhler & Milstein, 1975).
In the decades since, monoclonal antibodies have proven indispensable to the field of immunotherapy as cancer treatment. The domains of monoclonal antibody use that have proven successful so far are those of radioimmunotherapy (RIT) and immune checkpoint therapy (ICT). In radioimmunotherapy, monoclonal antibodies are paired with a radioactive payload (drug). These antibodies then home in on cancerous cell lineages and deliver the chemical dose. The efficacy of radioimmunotherapy has been clearly documented in the treatment of small-size tumors, especially those that are rapidly accessible to the injected antibody, such as bone marrow (Morschhauser et al., 2008). Optimal tumor targeting requires a high tumor uptake and a low retention of radioactivity in normal tissues, especially in the blood. Greater efficacy has been established using pre-targeting RIT techniques, which involve introducing the cancer-specific antibodies 2 to 4 days prior to payload injection; then, upon injection, the radioactive agent can rapidly bind to the pre-localized antibody (Gautherot et al., 1997; Paganelli et al., 2001).
More recently, antibodies have been used to bind to molecules involved in T-cell regulation to remove inhibitory pathways that block T-cell responses. Most cancers stimulate regulatory pathways that exist to terminate immune responses. Some of the most ubiquitous immune checkpoint inhibitors (ICIs) include the programmed cell death protein 1/programmed cell death ligand 1 (PD-1/PD-L1) and cytotoxic T lymphocyte-associated antigen-4 (CTLA-4) (Qin et al., 2019). When these regulatory checkpoints are activated, most immune cells are incapacitated and are consequently unable to initialize a formal attack on tumors. This inhibition occurs because CTLA-4 blocks the B7 receptor on the T cell that usually acts as a costimulatory molecule that induces immune response. Monoclonal antibodies have been used to localize and bind the inducers of these inhibitory pathways, thus preventing them from stimulating immune cell dormancy. This relatively new approach has been referred to as immune checkpoint therapy. Elevated PD-L1 expression in Hepatocellular carcinoma (HCC) has been associated with poorer prognosis in HCC patients (Wu et al., 2009). More recently, 90 HCC patients with PD-L1 expression in peritumoral hepatocytes (liver cells) were demonstrated to have a significantly higher risk of cancer recurrence or metastasis and cancer-related death (Dai et al., 2017). This clinical data further supports that PD-L1 is an important mediator in the progression of cancers and an important target in the anti-tumor therapy for liver cancer.
Limitations
Impediments to the use of immunotherapy as a standard cancer treatment include the failure of host cells to identify tumor antigens and the presence of negative immunoregulatory mechanisms (Waldmann, 2003). These mechanisms include Interleukin 2 (IL-2) mediated activation induced cell death (AICD), which leads to death of the tumor specific T-cells that are attempting to defend against cancer insurgence.
Despite some ICIs demonstrating effectiveness against certain tumor types, the majority of patients still show adaptive resistance, meaning that the cancer is able to regrow after treatment. Further identification of other vital checkpoint inhibitors whose incapacitation may wholly extinguish tumor resurgence provides a potential avenue for continued research (Qin et al., 2019). Currently, new immune checkpoints such as lymphocyte activation gene-3 (LAG-3), T cell immunoglobulin and mucin-domain-containing-3 (TIM-3) are being researched as potential drug targeting.
For solid cancers, RIT has not been as successful due to lower radio-sensitivity, difficult penetration of the antibody into the tumor, and potential excessive radiation to normal tissues (Frampas et al., 2013). Monoclonal antibodies have just begun to demonstrate effectiveness as a form of treatment, but further research promises an opportunity to harness this treatment as a standard therapy in the fight against cancer.
Antibody drug conjugates
One targeted cell therapy method for treating cancer is the use of Antibody-drug conjugates (ADCs). These drugs, which are composed of monoclonal antibodies linked to an anti-cancer drug, target specific antigen proteins found nearly exclusively on tumor cells. Therefore, the drugs ignore healthy cells that do not have the target antigen (Hamilton, 2015). Once the antibody binds to the target antigen, the cancer cell absorbs the ADC and it is transported to an internal cellular compartment called a lysosome. Under acidic conditions in the lysosome, the linkage between the antibody and drug is cleaved, allowing the drug to disrupt the cell’s DNA or prevent microtubule polymerization, thus inducing cell death (Hamilton, 2015).
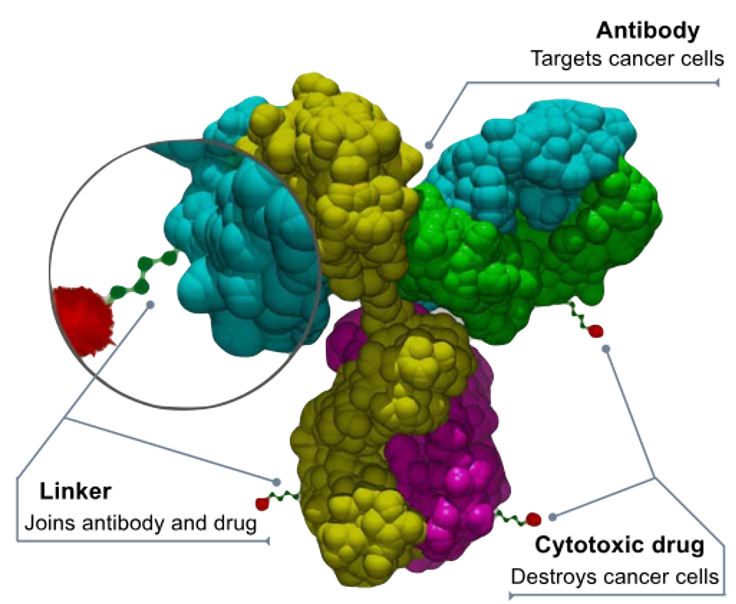
Figure 3: The structure of an antibody-drug conjugate. (Source: Bioconjugator, Wikimedia Commons)
Some ADCs have already demonstrated incredible efficacy and selectivity in targeting and eliminating tumor cells, while simultaneously lacking some of the strong negative effects of standard chemotherapy. Many ADCs make use of chemotherapeutic agents that would otherwise have been too toxic for direct use. For example, DiJoseph and colleagues were able to produce a CD-22 monoclonal antibody to deliver calicheamicin, which could be used to treat B-cell lymphoma lines (DiJoseph et. al, 2004). Calicheamicin is more than 4000 times more toxic than the standard doxorubicin used in chemotherapy and thus cannot be used alone (Kumar et. at, 2017). However, when combined with an anti-CD-22 monoclonal antibody through an acid-labile linker, the drug was found to achieve near total tumor death at a dose of 160 μg/kg of tumor, as compared to the 7.5 mg/kg required for the unconjugated drug. This dosage fell well within the range of tolerance for mice, which could handle doses up to 240 μg/kg (DiJoseph et. al, 2004). Similarly, Burris and colleagues discovered that the trastuzumab-DM1 antibody drug conjugate led to significant reduction of tumor growth for patients with human epidermal growth factor receptor 2 (HER2)-positive breast cancer (Burris et. al, 2011). The group found that 25.9% of patients experienced at least some tumor shrinkage when treated with the trastuzumab-DM1 ADC, with a further 49% experiencing no further growth of the cancer. Additionally, of the 112 patients in the study, only six patients required dose reduction from the 3.6 mg/kg prescribed, and no cardiotoxicity (damage to the heart) was detected (Burris et. al, 2011). Both of these studies demonstrate the potential benefits of ADCs as a targeted cancer treatment.
Cancer vaccines
Cancer vaccines, or immunotherapeutics, are another promising strategy for treating cancerous tumors (Apostopoulos, 2019). Some cancer vaccines are preventative, targeting cancer types that tend to develop as a result of viral infection; for example, the human papillomavirus (HPV) vaccine trains the immune system to mount an appropriate response to an HPV infection without developing into cervical or head and neck cancer (Dunn, n.d.). Other cancer vaccines are therapeutic, introducing either tumor-specific antigens or viral antigens (in the case of virally-induced cancers) to a cancer patient, directing immune response to the associated tumor (Dunn, n.d.). Sipuleucel-T is one such therapeutic cancer vaccine which presents prostatic acid phosphatase (PAP), an antigen specific to prostate cancer, to the immune system for targeting (Dunn, n.d.). Other commonly targeted tumor-associated antigens include mucin 1 for adenocarcinomas and human epidermal growth factor receptor 1 (HER2/neu) and insulin-like growth factor receptor-1 (IGF1R) for rhabdomyosarcoma (Apostopoulos, 2019).
A target of some cancer vacines are neoantigens, which are proteins produced only by tumor cells as a result of immunogenic mutation combinations common to certain cancer types, are an additional target for cancer vaccines (Apostopoulos, 2019; Dunn, n.d.). Because neoantigens are specific to cancer cells rather than normal cells, yet broad in their presentation in multiple cancers, they provide a promising target for immunotherapy. One of the greatest challenges to cancer vaccines as a form of immunotherapy is that the tumor microenvironment is often immunosuppressive; that is, cancers can hijack local immune cells to prevent them from mounting an attack on the tumor (Apostopoulos, 2019). By further studying the mechanisms by which cancer cells avoid immune targeting, scientists can better tailor cancer vaccines to achieve maximum efficacy.
Other Therapies
Gene Therapies
Gene therapies can be used to make tumor cells more recognizable by T cells. One way is to activate the genes coding specific antigens, which would make the tumor cell more immunogenic. During chemotherapy, patients are at risk of damaging their bone marrow. For this reason, scientists discovered a cancer gene therapy that would introduce a severely drug-resistant gene into the patient’s bone marrow as a protective measure. This gene helps synthesize a protein that efficiently removes the cytotoxins produced by chemotherapy, helping patients tolerate stronger doses of chemotherapy without experiencing adverse cytotoxic effects. Scientists have also thought of using cancer gene therapies to replace oncogenes, genes that when mutated can create a cancer phenotype, and with tumor suppressor genes, genes that help control cell growth; this method will likely continue to develop as additional oncogenes are identified and characterized.
Another prominent field of future cancer gene therapy research involves the use of viral vectors, which alter genes by using viruses as a way to efficiently control tumor cell growth. Because of this delivery method, viral vectors have proved to be more successful in their gene transfer in cancer cells. In addition, they are able to diffuse well throughout the malignant space. A challenge of these therapies is the fact that they are unable to target distinct tumor cells unless they have ligands connected to their DNA.
Hormone Therapy
Hormone therapy is a type of cancer treatment that slows or stops the growth of cancers that require hormones to grow, including certain breast, ovarian, and prostate cancers (“Hormone Therapy for Cancer,” 2015). Oftentimes, these cancer cells have hormone receptor proteins on their surface, to which hormones naturally made in the body can bind. These hormones, such as estrogen and progesterone, promote cancer cell growth and proliferation when they bind to the hormone receptors (“Hormone Therapy for Cancer Treatment,” n.d.). Thus, by depriving the cancer cells of a respective hormone, growth may be slowed or stopped altogether.
There are a few types of hormone therapies that achieve this goal, including drugs that block hormone receptors on cancer cells and others that prevent glands from making and secreting hormones in the first place (“Hormone Therapy for Cancer Treatment,” n.d.). Alternatively, doctors may surgically remove the glands that produce a given hormone depending on the type of cancer a patient has (“Hormone Therapy for Cancer Treatment,” n.d.). The specific type of hormone therapy administered to a patient depends on the type of cancer, the size of the tumor, the patient’s age, and the presence of hormone receptors on the cancer cells. Moreover, hormone therapy may be given before, concurrently with, or after other treatments depending on the patient’s needs. For instance, hormone therapy administered before surgery to remove a tumor can increase the likelihood of a successful procedure. On the other hand, hormone therapy administered after such a surgery can help prevent the cancer from relapsing (“Hormone Therapy for Cancer Treatment,” n.d.).
Despite the benefits of hormone therapy, it is not effective against all types of breast, ovarian, and prostate cancers. For instance, triple-negative breast cancer tests negative for estrogen and progesterone receptors, and also tests negative for excess HER2 protein, which promotes growth in other forms of breast cancer (“Triple-Negative Breast Cancer,” 2020). Without hormone receptors or excess HER2 protein to target, hormone and HER2 therapies are ineffective and other treatments are needed.
Precision medicine
The rise of precision medicine in oncology, or individualized approaches to treating a patient’s cancer, offers a promising therapeutic outlook. Currently, less than 25% of people with the most common cancers receive any form of precision therapy, but rapidly progressing research in this field aims to increase access to these individualized treatments (Boehm et al., 2021). One topic of particular interest is the Polygenic Risk Score (PRS)—a calculation which takes into account all known genetic and environmental risk factors to predict an individual’s likelihood of getting a certain disease (“Polygenic Risk,” 2019). Since cancer is caused by a multitude of factors that may differ among individuals, such an assessment may prove vital in determining preventative and therapeutic measures best suited for each patient (“Polygenic Risk,” 2019).
A group of researchers from the Wellcome Sanger Institute in the UK and the Broad Institute in Cambridge, Massachusetts is investigating a broader approach to tailoring individualized treatments (Boehm et al., 2021). This team is leading a project called the Cancer Dependency Map, which has an aim of evaluating every possible gene and drug perturbation in every known type of cancer based on laboratory data (Boehm et al., 2021). A highly ambitious undertaking, such a database would equip researchers and healthcare professionals with the knowledge to select the best possible drug to treat each patient’s cancer based on their unique genetic vulnerabilities.
Conclusion
From this overview of the various types of cancer treatments, it is evident that the field of drug development in oncology is incredibly complex and rapidly evolving. In this series, the history, mechanisms, and processes by which this occurs will continue to be explored. It is our hope that the series will serve as a comprehensive “one stop shop” for understanding this multifaceted field.
References
- Alkaloids. (2014, November 19). Chemistry LibreTexts. https://chem.libretexts.org/Courses/University_of_California_Davis/UCD_Chem_231A%3A_Methods_of_Organic_Synthesis/Under_construction/1_Ideas/Alkaloids
- Apostolopoulos, V. (2019). Cancer Vaccines: Research and Applications. Cancers, 11(8). https://doi.org/10.3390/cancers11081041
- Bakker, J. L., Wever, K., van Waesberghe, J. H., Beeker, A., Meijers-Heijboer, H., Konings, I. R., & Verheul, H. M. W. (2015). What is the benefit of treatment with multiple lines of chemotherapy for patients with metastatic breast cancer? A retrospective cohort study. Cancer Epidemiology, 39(6), 848–853. https://doi.org/10.1016/j.canep.2015.09.010
- Boehm, J. S., Garnett, M. J., Adams, D. J., Francies, H. E., Golub, T. R., Hahn, W. C., Iorio, F., McFarland, J. M., Parts, L., & Vazquez, F. (2021). Cancer research needs a better map. Nature, 589(7843), 514–516. https://doi.org/10.1038/d41586-021-00182-0
- Brentjens, R. J., Davila, M. L., Riviere, I., Park, J., Wang, X., Cowell, L. G., Bartido, S., Stefanski, J., Taylor, C., Olszewska, M., Borquez-Ojeda, O., Qu, J., Wasielewska, T., He, Q., Bernal, Y., Rijo, I. V., Hedvat, C., Kobos, R., Curran, K., … Sadelain, M. (2013). CD19-Targeted T Cells Rapidly Induce Molecular Remissions in Adults with Chemotherapy-Refractory Acute Lymphoblastic Leukemia. Science Translational Medicine, 5(177), 177ra38-177ra38. https://doi.org/10.1126/scitranslmed.3005930
- Breslin, S. (2007). Cytokine-Release Syndrome: Overview and Nursing Implications. Clinical Journal of Oncology Nursing, 11(0), 37–41. https://doi.org/10.1188/07.CJON.S1.37-42
- Brookes, P. (1990). The early history of the biological alkylating agents, 1918–1968. Mutation Research/Fundamental and Molecular Mechanisms of Mutagenesis, 233(1), 3–14. https://doi.org/10.1016/0027-5107(90)90145-T
- Burris, H. A., Rugo, H. S., Vukelja, S. J., Vogel, C. L., Borson, R. A., Limentani, S., Tan-
- Cancer Statistics. (2020, September 25). National Cancer Institute. https://www.cancer.gov/about-cancer/understanding/statistics.
- Cancer Vaccines: Preventive and Therapeutic – Cancer Research Institute (CRI). (n.d.). Retrieved February 12, 2021, from https://www.cancerresearch.org/immunotherapy/treatment-types/cancer-vaccines
- Carpenito, C., Milone, M. C., Hassan, R., Simonet, J. C., Lakhal, M., Suhoski, M. M., Varela-Rohena, A., Haines, K. M., Heitjan, D. F., Albelda, S. M., Carroll, R. G., Riley, J. L., Pastan, I., & June, C. H. (2009). Control of large, established tumor xenografts with genetically retargeted human T cells containing CD28 and CD137 domains. Proceedings of the National Academy of Sciences, 106(9), 3360–3365. https://doi.org/10.1073/pnas.0813101106
- Chan, K. S., Koh, C. G., & Li, H. Y. (2012). Mitosis-targeted anti-cancer therapies: where they stand. Cell death & disease, 3(10), e411-e411.
- Chiu, E., Krop, I. E., Michaelson, R. A., Girish, S., Amler, L., Zheng, M., Chu, Y.-W., Klencke, B., & O’Shaughnessy, J. A. (2011). Phase II Study of the Antibody Drug Conjugate Trastuzumab-DM1 for the Treatment of Human Epidermal Growth Factor Receptor 2 (HER2) –Positive Breast Cancer After Prir HER2-Directed Therapy. Journal of Clinical Oncology, 29(4), 398–405. https://doi.org/10.1200/JCO.2010.29.5865
- Colvin, M. (2003). Alkylating Agents. Holland-Frei Cancer Medicine. 6th Edition. https://www.ncbi.nlm.nih.gov/books/NBK12772/
- D’Andrea, A. D., & Haseltine, W. A. (1978). Sequence specific cleavage of DNA by the antitumor antibiotics neocarzinostatin and bleomycin. Proceedings of the National Academy of Sciences, 75(8), 3608-3612.
- Dai, X., Xue, J., Hu, J., Yang, S., Chen, G. G., Lai, P. B. S., Yu, C., Zeng, C., Fang, X., Pan, X., & Zhang, T. (2017). Positive Expression of Programmed Death Ligand 1 in Peritumoral Liver Tissue is Associated with Poor Survival after Curative Resection of Hepatocellular Carcinoma. Translational Oncology, 10(4), 511–517. https://doi.org/10.1016/j.tranon.2017.03.009
- DiJoseph, J. F., Armellino, D. C., Boghaert, E. R., Khandke, K., Dougher, M. M., Sridharan, L., Kunz, A., Hamann, P. R., Gorovits, B., Udata, C., Moran, J. K., Popplewell, A. G., Stephens, S., Frost, P., & Damle, N. K. (2004). Antibody-targeted chemotherapy with CMC-544: A CD22-targeted immunoconjugate of calicheamicin for the treatment of B-lymphoid malignancies. Blood, 103(5), 1807–1814. https://doi.org/10.1182/blood-2003-07-2466
- Dominguez-Brauer, C., Thu, K. L., Mason, J. M., Blaser, H., Bray, M. R., & Mak, T. W. (2015). Targeting mitosis in cancer: emerging strategies. Molecular cell, 60(4), 524-536.
- Eshhar, Z., Waks, T., GROSSt, G., & Schindler, D. G. (1993). Specific activation and targeting of cytotoxic lymphocytes through chimeric single chains consisting of antibody-binding domains and the y or C subunits of the immunoglobulin and T-cell receptors. Proc. Natl. Acad. Sci. USA, 5.
- Etoposide. (n.d.). Retrieved March 17, 2021, from https://go.drugbank.com/drugs/DB00773
- Frampas, E., Rousseau, C., Bodet-Milin, C., Barbet, J., Chatal, J.-F., & Kraeber-Bodéré, F. (2013). Improvement of Radioimmunotherapy Using Pretargeting. Frontiers in Oncology, 3. https://doi.org/10.3389/fonc.2013.00159
- Galm, U., Hager, M. H., Van Lanen, S. G., Ju, J., Thorson, J. S., & Shen, B. (2005). Antitumor antibiotics: bleomycin, enediynes, and mitomycin. Chemical Reviews, 105(2), 739-758.
- Gautherot, E., Bouhou, J., Doussal, J.-M. L., Manetti, C., Martin, M., Rouvier, E., & Barbet, J. (1997). Therapy for colon carcinoma xenografts with bispecific antibody‐targeted, iodine‐131‐labeled bivalent hapten. Cancer, 80(S12), 2618–2623. https://doi.org/10.1002/(SICI)1097-0142(19971215)80:12+<2618::AID-CNCR37>3.0.CO;2-D
- Hamilton, G. S. (2015). Antibody-drug conjugates for cancer therapy: The technological and regulatory challenges of developing drug-biologic hybrids. Biologicals, 43(5), 318–332. https://doi.org/10.1016/j.biologicals.2015.05.006
- Hartmann, J., Schüßler‐Lenz, M., Bondanza, A., & Buchholz, C. J. (2017). Clinical development of CAR T cells—Challenges and opportunities in translating innovative treatment concepts. EMBO Molecular Medicine, 9(9), 1183–1197. https://doi.org/10.15252/emmm.201607485
- Hormone Therapy For Cancer Treatment—Health Encyclopedia—University of Rochester Medical Center. (n.d.). Retrieved March 17, 2021, from https://www.urmc.rochester.edu/encyclopedia/content.aspx?contenttypeid=85&contentid=p07236
- Hormone Therapy for Cancer—National Cancer Institute (nciglobal,ncienterprise). (2015, April 29). [CgvArticle]. https://www.cancer.gov/about-cancer/treatment/types/hormone-therapy
- How Immunotherapy Is Used to Treat Cancer. (2019, December 27). American Cancer Society. https://www.cancer.org/treatment/treatments-and-side-effects/treatment-types/immunotherapy/what-is-immunotherapy.html
- How Radiation Therapy Is Used to Treat Cancer. (2019, December 27). American Cancer Society. https://www.cancer.org/treatment/treatments-and-side-effects/treatment-types/radiation/basics.html
- Immunotherapy for Cancer (nciglobal,ncienterprise). (2019, September 24). National Cancer Institute. https://www.cancer.gov/about-cancer/treatment/types/immunotherapy
- Immunotherapy: Side Effects, Risks & Benefits. (2019, March 25). Cancer Therapy Advisor. https://www.cancertherapyadvisor.com/home/tools/fact-sheets/immunotherapy-side-effects-risks-and-benefits/
- Jack A. Roth, Richard J. Cristiano, Gene Therapy for Cancer: What Have We Done and Where Are We Going?, JNCI: Journal of the National Cancer Institute, Volume 89, Issue 1, 1 January 1997, Pages 21–39, https://doi.org/10.1093/jnci/89.1.21
- Jacobson, C. A., & Ritz, J. (2011). Time to put the CAR-T before the horse. Blood, 118(18), 4761–4762. https://doi.org/10.1182/blood-2011-09-376137
- Kalos, M., Levine, B. L., Porter, D. L., Katz, S., Grupp, S. A., Bagg, A., & June, C. H. (2011). T Cells with Chimeric Antigen Receptors Have Potent Antitumor Effects and Can Establish Memory in Patients with Advanced Leukemia. Science Translational Medicine, 3(95), 95ra73-95ra73. https://doi.org/10.1126/scitranslmed.3002842
- Kochenderfer, J. N., Dudley, M. E., Kassim, S. H., Somerville, R. P. T., Carpenter, R. O., Stetler-Stevenson, M., Yang, J. C., Phan, G. Q., Hughes, M. S., Sherry, R. M., Raffeld, M., Feldman, S., Lu, L., Li, Y. F., Ngo, L. T., Goy, A., Feldman, T., Spaner, D. E., Wang, M. L., … Rosenberg, S. A. (2015). Chemotherapy-Refractory Diffuse Large B-Cell Lymphoma and Indolent B-Cell Malignancies Can Be Effectively Treated With Autologous T Cells Expressing an Anti-CD19 Chimeric Antigen Receptor. Journal of Clinical Oncology, 33(6), 540–549. https://doi.org/10.1200/JCO.2014.56.2025
- Kochenderfer, J. N., Wilson, W. H., Janik, J. E., Dudley, M. E., Stetler-Stevenson, M., Feldman, S. A., Maric, I., Raffeld, M., Nathan, D.-A. N., Lanier, B. J., Morgan, R. A., & Rosenberg, S. A. (2010). Eradication of B-lineage cells and regression of lymphoma in a patient treated with autologous T cells genetically engineered to recognize CD19. Blood, 116(20), 4099–4102. https://doi.org/10.1182/blood-2010-04-281931
- Köhler, G., & Milstein, C. (1975). Continuous cultures of fused cells secreting antibody of predefined specificity. Nature, 256(5517), 495–497. https://doi.org/10.1038/256495a0
- Kumar, A., White, J., James Christie, R., Dimasi, N., & Gao, C. (2017). Chapter Twelve—Antibody-Drug Conjugates. In R. A. Goodnow (Ed.), Annual Reports in Medicinal Chemistry (Vol. 50, pp. 441–480). Academic Press. https://doi.org/10.1016/bs.armc.2017.08.002
- Lim, W. A., & June, C. H. (2017). The Principles of Engineering Immune Cells to Treat Cancer. Cell, 168(4), 724–740. https://doi.org/10.1016/j.cell.2017.01.016
- Longdom Publishing (Ed.). (2021). Anti-tumor Antibiotics. Journal of Cancer Science and Research.https://www.longdom.org/scholarly/antitumor-antibiotics-journals-articles-ppts-list-2978.html#:~:text=Anthracyclines%20are%20anti%2Dtumor%20antibiotics,for%20a%20variety%20of%20cancers.
- Mannion, E., Gilmartin, J. J., Donnellan, P., Keane, M., & Waldron, D. (2014). Effect of chemotherapy on quality of life in patients with non-small cell lung cancer. Supportive Care in Cancer: Official Journal of the Multinational Association of Supportive Care in Cancer, 22(5), 1417–1428. https://doi.org/10.1007/s00520-014-2148-9
- Maude, S. L., Frey, N., Shaw, P. A., Aplenc, R., Barrett, D. M., Bunin, N. J., Chew, A., Gonzalez, V. E., Zheng, Z., Lacey, S. F., Mahnke, Y. D., Melenhorst, J. J., Rheingold, S. R., Shen, A., Teachey, D. T., Levine, B. L., June, C. H., Porter, D. L., & Grupp, S. A. (2014). Chimeric Antigen Receptor T Cells for Sustained Remissions in Leukemia. New England Journal of Medicine, 371(16), 1507–1517. https://doi.org/10.1056/NEJMoa1407222
- Messerschmidt, J. L., Prendergast, G. C., & Messerschmidt, G. L. (2016). How Cancers Escape Immune Destruction and Mechanisms of Action for the New Significantly Active Immune Therapies: Helping Nonimmunologists Decipher Recent Advances. The Oncologist, 21(2), 233–243. https://doi.org/10.1634/theoncologist.2015-0282
- Morschhauser, F., Radford, J., Van Hoof, A., Vitolo, U., Soubeyran, P., Tilly, H., Huijgens, P. C., Kolstad, A., d’Amore, F., Diaz, M. G., Petrini, M., Sebban, C., Zinzani, P. L., van Oers, M. H. J., van Putten, W., Bischof-Delaloye, A., Rohatiner, A., Salles, G., Kuhlmann, J., & Hagenbeek, A. (2008). Phase III Trial of Consolidation Therapy With Yttrium-90–Ibritumomab Tiuxetan Compared With No Additional Therapy After First Remission in Advanced Follicular Lymphoma. Journal of Clinical Oncology, 26(32), 5156–5164. https://doi.org/10.1200/JCO.2008.17.2015
- Munzone, E., & Colleoni, M. (2015). Clinical overview of metronomic chemotherapy in breast cancer. Nature Reviews Clinical Oncology, 12(11), 631–644. https://doi.org/10.1038/nrclinonc.2015.131
- Nicolaou, K. C., & Dai, W. M. (1991). Chemistry and biology of the enediyne anticancer antibiotics. Angewandte Chemie International Edition in English, 30(11), 1387-1416.
- Paganelli, G., Bartolomei, M., Ferrari, M., Cremonesi, M., Broggi, G., Maira, G., Sturiale, C., Grana, C., Prisco, G., Gatti, M., Caliceti, P., & Chinol, M. (2001). Pre-Targeted Locoregional Radioimmunotherapy with 90Y-biotin in Glioma Patients: Phase I Study and Preliminary Therapeutic Results. Cancer Biotherapy and Radiopharmaceuticals, 16(3), 227–235. https://doi.org/10.1089/10849780152389410
- Palliative care in children with cancer: Which child and when? – PubMed. (n.d.). Retrieved February 24, 2021, from https://pubmed.ncbi.nlm.nih.gov/15263058/
- Pharmacokinetics of metronomic chemotherapy: A neglected but crucial aspect | Nature Reviews Clinical Oncology. (n.d.). Retrieved February 24, 2021, from https://www.nature.com/articles/nrclinonc.2016.64
- Plant Alkaloids. (2014, December 19). [Text]. Australian Government – Cancer Australia; Cancer Australia. https://www.edcan.org.au/edcan-learning-resources/supporting-resources/antineoplastic-agents/classification/plant-alkaloids
- Polygenic risk: What’s the score? (2019). Nature Research. https://www.nature.com/articles/d42473-019-00270-w
- Prendergast, G. C., & Jaffee, E. M. (Eds.). (2007). Cancer immunotherapy: immune suppression and tumor growth. Academic Press/Elsevier.
- Qin, S., Xu, L., Yi, M., Yu, S., Wu, K., & Luo, S. (2019). Novel immune checkpoint targets: Moving beyond PD-1 and CTLA-4. Molecular Cancer, 18(1), 155. https://doi.org/10.1186/s12943-019-1091-2
- Scharovsky, O. G., Mainetti, L. E., & Rozados, V. R. (2009). Metronomic chemotherapy: Changing the paradigm that more is better. Current Oncology, 16(2), 7–15.
- Sharma, P., & Allison, J. P. (2015). The future of immune checkpoint therapy. Science, 348(6230), 56–61. https://doi.org/10.1126/science.aaa8172
- Shewach, D. S., & Kuchta, R. D. (2009). Introduction to Cancer Chemotherapeutics. Chemical Reviews, 109(7), 2859–2861. https://doi.org/10.1021/cr900208x
- Sinha, B. K. (1995). Topoisomerase inhibitors. Drugs, 49(1), 11-19.
- Surgery for Cancer (nciglobal,ncienterprise). (2015, April 29). National Cancer Institute. https://www.cancer.gov/about-cancer/treatment/types/surgery
- Triple-Negative Breast Cancer: Overview, Treatment, and More. (2020, September 21). Breastcancer.Org. https://www.breastcancer.org/symptoms/diagnosis/trip_neg
- Turtle, C. J., Hanafi, L.-A., Berger, C., Gooley, T. A., Cherian, S., Hudecek, M., Sommermeyer, D., Melville, K., Pender, B., Budiarto, T. M., Robinson, E., Steevens, N. N., Chaney, C., Soma, L., Chen, X., Yeung, C., Wood, B., Li, D., Cao, J., … Maloney, D. G. (2016). CD19 CAR–T cells of defined CD4+:CD8+ composition in adult B cell ALL patients. The Journal of Clinical Investigation, 126(6), 2123–2138. https://doi.org/10.1172/JCI85309
- Types of Chemotherapy Drugs | SEER Training. (n.d.). Retrieved March 17, 2021, from https://training.seer.cancer.gov/treatment/chemotherapy/types.html
- Types of Oncologists. (2018, March). Cancer.Net. https://www.cancer.net/navigating-cancer-care/cancer-basics/cancer-care-team/types-oncologists
- Understanding Immunotherapy. (2020, May). Cancer.Net. https://www.cancer.net/navigating-cancer-care/how-cancer-treated/immunotherapy-and-vaccines/understanding-immunotherapy
- Verweij, J., & Pinedo, H. M. (1990). Mitomycin C: mechanism of action, usefulness and limitations. Anticancer Drugs, 1(1), 5-13.
- Waldmann, T. A. (2003). Immunotherapy: Past, present and future. Nature Medicine, 9(3), 269–277. https://doi.org/10.1038/nm0303-269
- What is Cancer Surgery? (2019, May). Cancer.Net. https://www.cancer.net/navigating-cancer-care/how-cancer-treated/surgery/what-cancer-surgery
- What Is QOL? | ISOQOL. (n.d.). Retrieved March 22, 2021, from https://www.isoqol.org/what-is-qol/
- Wu, K., Kryczek, I., Chen, L., Zou, W., & Welling, T. H. (2009). Kupffer Cell Suppression of CD8 + T Cells in Human Hepatocellular Carcinoma Is Mediated by B7-H1/Programmed Death-1 Interactions. Cancer Research, 69(20), 8067–8075. https://doi.org/10.1158/0008-5472.CAN-09-0901
- Xu, F., Jin, T., Zhu, Y., & Dai, C. (2018). Immune checkpoint therapy in liver cancer. Journal of Experimental & Clinical Cancer Research, 37(1), 110. https://doi.org/10.1186/s13046-018-0777-4
Related Posts
Targeting crucial molecules used by coronaviruses to infect cells may lead to a broader antiviral treatment
Figure 1: Researchers have identified crucial molecules utilized by the...
Read MoreBacterial Treatment of Cancer
Abstract: Cancer treatment has long been an engaging field of...
Read MoreStaphylococcus epidermidis capable of 6-HAP production reduce host UV-induced tumors
Figure 1: The human microbiome is the collection of microorganisms...
Read MoreThe Neurobiological Basis of Autism
For more neuroscience news, check out the UnknownNow, a student...
Read MoreThe Pathway to Sonogenetics: Understanding the Mechanical Nature of the Ultrasound Stimulation Mechanism in C. Elegans
Figure 1: C. elegans in Agar under Microscope Magnification Source:...
Read MoreThe Looming Pandemic of Antimicrobial Resistance
Extensive and irresponsible use of antimicrobial drugs is one of...
Read MoreDina Rabadi, Sarah Matatov, Carolina Guerrero, Nina Klee,Whitney Nicanor Mabwi, Bamlak Messay, Yu Quan Ng, Melanie Prakash, Andrew Sasser, Jillian Troth